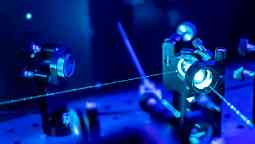
Optical Sciences Centre
We conduct research addressing scientific and technological challenges in the domain of optical sciences spanning classical and quantum photonics, light–matter interactions, nanotechnology, biomedical and biosciences, quantum gases and quantum materials.
The Optical Sciences Centre is dedicated to pursuing excellence and impact through world-leading research, focusing on both fundamental and applied areas of optical sciences and related areas. Led by Professor David Moss (Director) and Professor Chris Vale and Professor Saulius Juodkazis (Deputy Directors), our centre incorporates staff from the previous Centre for Micro-Photonics and Centre for Quantum and Optical Science to create one of the largest optical research facilities in Australia.
Our vision is to conduct research and development across the full spectrum of research engagement and translation. From fundamental studies in the way light interacts with matter, through to the development of technologies for market, we foster cross-disciplinary collaborations that address scientific challenges in fields encompassing classical and quantum photonics, light-matter interactions, nanotechnology, biomedical and biosciences, quantum gases and quantum materials.
-
-
-
Our study options
We offer opportunities for students wishing to undertake a PhD, masters, honours or undergraduate degree.
-
- Technology
Australian researchers record world’s fastest internet speed from a single optical chip
Researchers have recorded the world’s fastest internet speed from a single optical chip of 44.2 Terabits per second.Friday 22 May 2020
Our publications
The Optical Sciences Centre is a new state-of-the-art research facility with a range of researcher experience, including from the Centre for Micro-Photonics and the Centre for Quantum and Optical Science.
Read publications from the Centre for Micro-Photonics researchers
Read publications from the Centre for Quantum and Optical Science researchers
Centre news
-
- Technology
- Science
Swinburne-led research team demonstrates world’s fastest optical neuromorphic processor
An international team of researchers has demonstrated the world’s fastest and most powerful optical neuromorphic processor for artificial intelligence, which is capable of processing ultra-large scale data
Thursday 07 January 2021 -
- Science
New optical pulse seen in micro-combs may unlock practical applications
A new class of microcomb pulse may lead to pocket sized ultra-precise optical clocks able to replace GPS and other applications.Tuesday 12 March 2019 -
- Science
Quantum optical micro-combs enable breakthroughs in quantum world
Compact quantum devices could bring the vision of powerful optical quantum computers for everyday use closer than ever before.Monday 25 February 2019 -
- Science
Nobel-winning science is key to Australian ultra-fast laser physics research
Swinburne researchers are using ultra-fast laser technique developed by 2018 Nobel Prize for Physics winnersThursday 04 October 2018
Find more news articles by visiting the Centre for Quantum and Optical Science newsroom and the Centre for Micro-Photonics newsroom.
Explore other research centres
-
Centre for Astrophysics and Supercomputing
-
Centre for Design Innovation
-
Centre for Forensic Behavioural Science
-
Centre for Social Impact Swinburne
-
Centre for Human Psychopharmacology
-
Centre for Mental Health
-
Centre for the New Workforce
-
Centre for Sustainable Infrastructure and Digital Construction
-
Centre for Transformative Innovation
-
Centre for Urban Transitions
-
Centre for Global Health and Equity
-
Centre for Transformative Media Technologies
-
Centre for Quantum Technology Theory
-
Centre for Translational Atomaterials
How you can reach us
Location
Optical Sciences Centre
Swinburne University of Technology
John Street
Hawthorn VIC 3122
Australia
Postal address
Optical Sciences Centre
Swinburne University of Technology
Mail H74
PO Box 218
Hawthorn VIC 3122
Australia
Contact the Optical Sciences Centre
There are many ways to engage with us. If your organisation is dealing with a complex problem, get in touch to discuss how we can work together to provide solutions. Call us on +61 3 9214 8096 or email osc@swinburne.edu.au.